[ad_1]
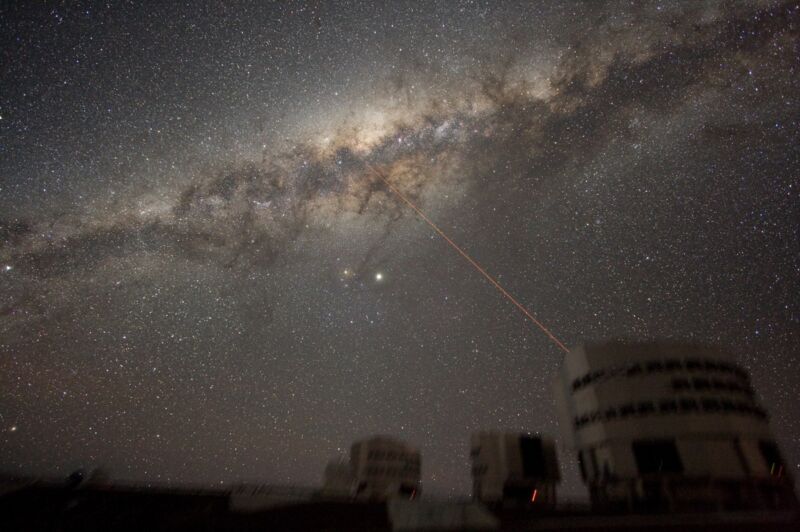
The 2020 Nobel Prize in Physics has been awarded to Roger Penrose “for the discovery that the formation of black holes is a robust prediction of the general theory of relativity.” He shares it with Reinhard Genzel and Andrea Ghez “for the discovery of a supermassive compact object at the center of our galaxy.”
Penrose, Rouse Ball Professor Emeritus of Mathematics at Oxford University, will receive half of the 10 million Swedish crowns (more than $ 1.1 million) in prizes. It helped solidify the theoretical basis for black hole physics in the 1960s by providing the fundamental mathematical proof that black holes were a direct consequence of general relativity.
Genzel is acting director of the Max Planck Institute for Extraterrestrial Physics in Germany and a professor at the University of California, Berkeley, while Ghez is a professor at the University of California, Los Angeles. Each will receive a quarter of the prize money. Genzel and Ghez lead astronomy groups that have mapped the orbits of the stars closest to the center of our Milky Way, a region known as Sagittarius A *, giving us the best evidence to date that there is a supermassive black hole in the center of our galaxy. That work was greatly aided by the development of advanced adaptive optics tools to counteract the distorting effects of Earth’s atmosphere.
“The discoveries of this year’s laureates have broken new ground in the study of compact and supermassive objects,” said David Haviland, chairman of the Nobel Committee in Physics, in an official statement. But these exotic objects still raise many questions that beg for answers and motivate future research. Not only questions about its internal structure, but also questions about how to test our theory of gravity under extreme conditions in the immediate vicinity of a black hole. “
Dark stars
Black holes have a precursor in the “dark stars” hypothesized by John Michell in 1783 and Pierre-Simon LaPlace in 1796. In a 1783 paper in Philosophical Transactions of the Royal Society, Michell argued that, according to classical (Newtonian) mechanics , a star of approximately the same density as our Sun, but with a radius 500 times greater, would generate such a strong gravitational pull that the light itself would be trapped. LaPlace made similar calculations in his own 1799 paper.
Our modern concept of a black hole dates back to 1916, when Albert Einstein’s general theory of relativity was completely new and revolutionized our understanding of gravity. Einstein envisioned a spacetime that is curved, not flat, and therefore gravity is not so much a force as a spacetime that has been deformed by the presence of mass or energy. The amount of mass or energy present determines the degree of curvature and the more it curves, the stronger the gravitational pull. Since space and time are one, what happens to space also affects time: when space warps, time is stretched or compressed accordingly. Therefore, time slows down in direct proportion to the strength of a gravitational field, and the strength of that field depends on distance.
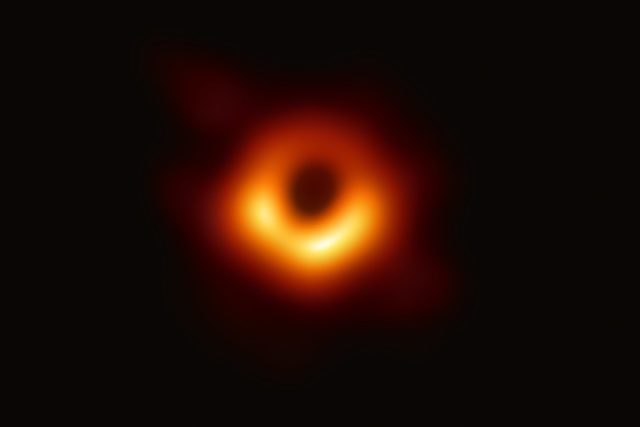
Einstein’s equations opened up a whole new realm of theoretical possibilities. A physicist named Karl Schwarzschild began toying with different solutions while under heavy gunfire at the front during World War I, right after Einstein published his seminal paper, his way of taking his mind off the horrors of war. Schwarzschild eventually hit a roadblock where the equations “exploded” and his work provided an early description of a black hole. (Robert Dicke coined the term in 1960, and John Wheeler later helped popularize it.) An extremely heavy mass can cut through a chunk of space to form a black hole, surrounded by an event horizon, a hypothetical point of no return beyond which nothing can escape (not even light). The greater the mass, the larger the black hole and the larger the diameter of its event horizon.
These exotic objects were initially considered by physicists to be purely theoretical, although Robert Oppenheimer and his student Hartland Snyder did some initial calculations showing that massive stars, many times more massive than our Sun, could dramatically collapse to form black holes. “The star, therefore, tends to shut itself off from any communication with a distant observer; only its gravitational field persists,” they concluded. However, the general consensus was that this was not a realistic model for something that could actually form in our universe. Then physicists discovered quasars in the 1960s, the brightest known objects in the universe. The source of all that radiation, the scientists concluded, had to be matter falling into a huge black hole. So black holes could be “real” after all.
Roger Penrose decided to tackle the problem of demonstrating how black holes could realistically form and then recalled the moment when he reached his key idea in the fall of 1964. While strolling through London while visiting a colleague, he envisioned a “trapped surface” : a two-dimensional surface that directs all light rays to an infinitely dense center, what we now call the singularity, where time and space end up inside a black hole. Penrose went on to demonstrate, using his Penrose diagrams of the same name, among other tools, that once such a trapped surface has been formed, under general relativity, nothing can stop the inevitable collapse towards the singularity.
Journey to the center of the Milky Way
However, establishing a solid theoretical basis for the existence of black holes was not the same as observing one directly. Our Milky Way is a flat disk that is approximately 100,000 light-years across, and our Sun is just one of hundreds of billions of stars it contains. Physicists had long thought that there might be a supermassive black hole at its center, bolstered by the discovery of radio waves emanating from that central region known as Sagittarius A *. So it seemed like an ideal candidate for further investigation.
But how, exactly, do you “observe” an object from which no light can escape? It must be done indirectly, by measuring the gravitational effects that such an object would exert on nearby objects, such as the orbits of nearby stars. This must be done with ground-based telescopes making observations in the near infrared, as any light in the optical spectrum would be obscured by interstellar gas and dust. The finer the scale at which these movements can be tracked, the easier it is to perform the necessary calculations.
Starting in the 1990s, Genzel’s team relied on the telescopes of the European Southern Observatory in Chile, most notably the Very Large Telescope array. Meanwhile, Ghez’s team relied on the Keck telescope in Hawaii.
The work was laborious, slow, and hampered by the turbulent effects of the Earth’s atmosphere. Genzel and Ghez and their respective teams developed a technique called “speckle imaging” to address that challenge. It involves taking several short, highly sensitive exposures of a given star and stacking that data to produce a sharper image. But this was only effective for the brightest stars orbiting Sagittarius A *, and it also took years to get the necessary information about the velocities of a handful of those stars.
The advent of adaptive optics in the late 1990s proved to be a game changer. This involves the use of a “guide star” as the first observation point, either a real star or an artificially created point source, which can be achieved by using a powerful laser to excite sodium atoms in the upper atmosphere. Once the position and brightness of the guide star have been determined, that information can be used to calculate the effects of atmospheric turbulence. This, in turn, allows astronomers to use rapidly deformable mirrors to compensate for the distortion.
The use of adaptive optics allows longer exposures, so more stars can be observed at a much greater image depth. The two teams were able to monitor the movement of about 30 bright stars near Sagittarius A * on a much shorter time scale. They were both able to image and analyze a particular star near the galactic center, S2, which completes an orbit in just under 16 years (compared to the 200 million years it takes for our Sun to complete its orbit around the center of the galaxy). Milky Way). and their data matched perfectly. Conclusion: the object in the center of the Milky Way is a supermassive black hole.
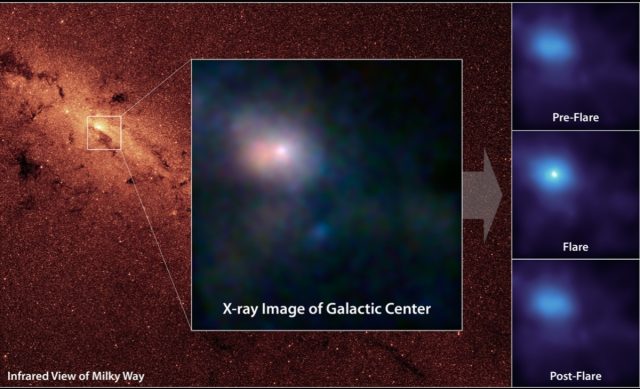
NASA / public domain
We are poised to make even more exciting discoveries about black holes in the future. For example, it is very likely that we will soon have a real image of the black hole at the center of our galaxy, courtesy of the Event Horizon Telescope, which made headlines last year for its impressive image of the black hole at the center of the galaxy. Messier 87 galaxy, about 55 million light years from Earth. And the ongoing LIGO / VIRGO collaboration continues to detect gravitational waves produced by black hole mergers, among other cosmic events.
“Research on the dark universe, which was once an exotic topic, is becoming increasingly common,” INFN’s Giovanni Losurdo, spokesman for Virgo Collaboration, said in a statement in response to the award announcement. “In fact, the gravitational wave discovery announced in 2016 was also the first direct detection of a black hole. Since then, Virgo and Ligo have detected dozens of binary black hole systems, allowing us to take a closer look at the physics of these objects are still partly mysterious and in the mechanisms of their formation. This year’s Nobel Prize encourages us to continue on the path already taken by our research. “
Editor’s Note: In the wake of last year’s awards, we started a discussion about the awards. The end result was that we decided to do a quicker coverage on award day and see if there were any facets of the work that deserved deeper coverage that could be done later. At this time, we don’t see anything about this year’s physics award to suggest that more detailed coverage would be informative to our readers.