[ad_1]
Computer simulation of a merger between two dense neutron stars. After fusion, a phase transition from ordinary hadronic matter (red-yellow) to quark matter (green) takes place.
In the first fractions of a second of our universe’s existence, the energy density was so incredibly high that there were no protons or neutrons, just a hot “quark soup” known as quark-gluon plasma (QGP). Physicists have successfully recreated this unique state of matter in high-energy laboratories, but those conditions are extremely rare in today’s cosmos. According to a new article in the journal Physical Review Letters, German physicists have run computer simulations indicating that a QGP could form immediately after a binary neutron star merger, and that it should produce a telltale and telltale signature on the waves. gravitational emanating from that event
“Compared to previous simulations, we have discovered a new signature in gravitational waves that is significantly clearer to detect,” said co-author Luciano Rezzolla of Goethe University in Frankfurt, Germany. “If this signature occurs in the gravitational waves that we will receive from future neutron star mergers, we would have clear evidence of the creation of quark-gluon plasma in the current universe.”
A hot and dense soup
The Quarks, the building blocks of subatomic particles, are linked by force-bearing gluons to form protons and neutrons. But in the extreme high-energy conditions of the early universe in its first microseconds of existence, that could not happen. Instead, the quarks and gluons were freely mixed in a dense soup, until things cooled down enough for the protons to condense from the QGP. Before the first second was over, the Universe had gone through its entire inflationary period, sowing the seeds for the large-scale structures we see today.
To create QGP in the lab, at the Brookhaven National Laboratory (RHIC) relativistic heavy ion collider and at CERN’s large hadron collider, physicists must reach temperatures hundreds of thousands of times higher than the sun. They do this through heavy ion collisions (using gold in RHIC, lead in the LHC) to generate the enormously high energies necessary to destroy the nucleon. As John Timmer of Ars explained in 2012:
The quarks and gluons that spill out from a proton collision tend to have nothing but empty space around them. In a heavy ion collision, the large number of nucleons that break at once means that, instead of flying into empty space, a given quark or gluon will have the opportunity to interact with those leaving the nearby nucleons. As a result, for a brief moment, collisions don’t look much like an explosion; instead, it is more like the boundaries between melting nucleons, leaving behind a sea of interacting quarks and gluons.
Physicists were surprised to discover in 2005 that QGP is not really a plasma gas in terms of its behavior. It is more similar to a superfluid, since there is practically no viscosity (resistance to friction); in fact, it is the most perfect liquid observed so far. Subsequent experiments revealed that particle collisions within the QGP spin faster (a measure of vorticity) than any other known fluid as well.
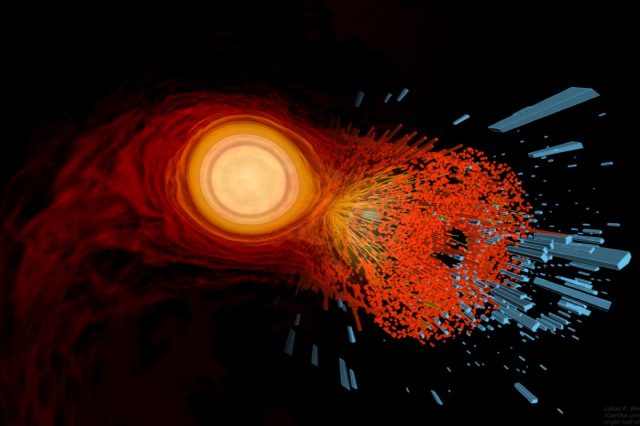
Lukas R. Weih and L. Rezzolla (GUF) / CERN
A revealing signature?
Where could we find a QGP in the universe today? Rezzolla and his GUF colleagues thought that a binary neutron star fusion might be a good candidate, and hypothesized that we could find evidence of a QGP in the gravitational waves produced by such a fusion, thanks to LIGO’s continued success.
LIGO detects gravitational waves through laser interferometry, using high-powered lasers to measure small changes in the distance between two objects located kilometers away. (A third detector went online at VIRGO in 2016.) Each instrument is so sensitive that it also picks up small ambient vibrations, like a rumbling load train or natural thermal vibrations in the detectors.
On September 14, 2015, at 5:51 AM EST, both detectors picked up signals within milliseconds of each other for the first time. The waveforms of those signals serve as an audio fingerprint, in this case evidence of two black holes spiraling inward and merging in a massive collision event, sending powerful shock waves through space-time. . That first detection hooked the collaboration of the 2017 Nobel Prize in Physics.
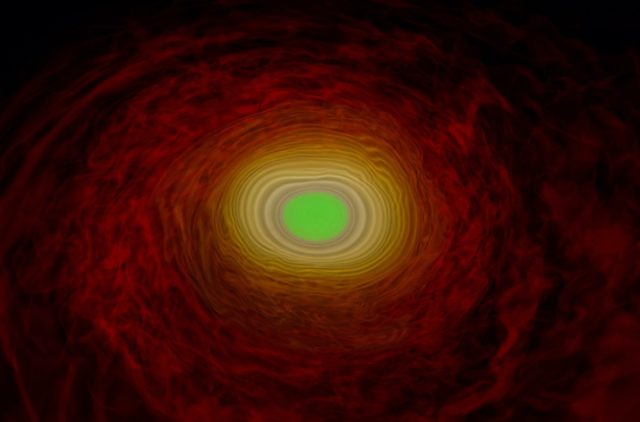
Lukas R. Weihh and Luciano Rezzola / GUF
Since then, there have been a series of additional gravitational wave detections, most notably the 2017 binary neutron star merger, backed by a simultaneous burst of gamma rays and signals across the rest of the electromagnetic spectrum. And last year LIGO / VIRGO observed five gravitational wave events in a single month. Three came from the black hole fusion, one was the second neutron star fusion, and another may have been the first instance of a black hole neutron star fusion. Therefore, the probability of detecting additional binary neutron star mergers in the future is strong.
But much of the focus on those early detections has been at the melting point; Gravitational wave recordings were not made beyond that. Rezzolla et al. He used computer simulations to model two merged neutron stars, focusing on the immediate aftermath of that merger. Those simulations showed that a phase transition would occur, so the extreme environment would cause the hadrons to melt and briefly create a QGP of quarks and gluons that mix freely. That transition should leave a clear and distinct signature on the gravitational wave signals emanating from that event.
Now we just have to wait for LIGO / VIRGO to detect another neutron star fusion, this time recording a bit more in order to capture a signal from that telltale phase transition.
DOI: Physical Review Letters2020. 10.1103 / PhysRevLett.124.171103 (About DOIs).
Listing image for Lukas R. Weih and L. Rezzolla (GUF) / CERN